13.4. Population biology — predator/parasitoid and prey/host abundance
Ecological interactions between an individual, its conspecifics, its predators and parasitoids (and other causes of mortality), and its abiotic habitat are fundamentally important aspects of population dynamics. Accurate estimation of population density and its regulation is at the heart of population ecology, biodiversity studies, conservation biology, and monitoring and management of pests. A range of tools are available to entomologists to understand the effects of the many factors that influence population growth and survivorship, including sampling methods, experimental designs, and manipulations and modeling programs.
Insects usually are distributed on a wider scale than investigators can survey in detail, and thus sampling must be used to allow extrapolation to the wider population. Sampling may be absolute, in which case all organisms in a given area or volume might be assessed, such as mosquito larvae per liter of water, or ants per cubic meter of leaf litter. Alternatively, relative measures, such as number of Collembola in pitfall trap samples, or micro-wasps per yellow pan trap, may be obtained from an array of such trapping devices (section 17.1). Relative measures may or may not reflect actual abundances, with variables such as trap size, habitat structure, and insect behavior and activity levels affecting “trappability” — the likelihood of capture. Measures may be integrated over time, for example a series of sticky, pheromone, or continuous running light traps, or instantaneous snap-shots such as the inhabitants of a submerged freshwater rock, the contents of a timed sweep netting, or the knock-down from an insecticidal fogging of a tree’s canopy. Instantaneous samples may be unrepresentative, whereas longer duration sampling can overcome some environmental variability.
Sampling design is the most important component in any population study, with stratified random designs providing power to interpret data statistically. Such a design involves dividing the study site into regular blocks (subunits) and, within each of these blocks, sampling sites are allocated randomly. Pilot studies can allow understanding of the variation expected, and the appropriate matching of environmental variables between treatments and controls for an experimental study. Although more widely used for vertebrate studies, mark-and-recapture methods have been effective for adult odonates, larger beetles, moths and, with fluorescent chemical dyes, smaller pest insects.
A universal outcome of population studies is that the expectation that the number and density of individuals grows at an ever-increasing rate is met very rarely, perhaps only during short-lived pest outbreaks. Exponential growth is predicted because the rate of reproduction of insects potentially is high (hundreds of eggs per mother) and generation times are short — even with mortality as high as 90%, numbers increase dramatically. The equation for such geometric or exponential growth is:
dN/dt = rN
where N is population size or density, dN/dt is the growth rate, and r is the instantaneous per capita rate of increase. At r = 0 rates of birth and death are equal and the population is static; if r < 0 the population declines; when r > 0 the population increases.
Growth continues only until a point at which some resource(s) become limiting, called the carrying capacity. As the population nears the carrying capacity, the rate of growth slows in a process represented by:
dN/dt = rN — rN2/K
in which K, representing the carrying capacity, contributes to the second term, called environmental resistance.
Although this basic equation of population dynamics underpins a substantial body of theoretical work, evidently natural populations persist in more narrowly fluctuating densities, well below the carrying capacity. Observed persistence over evolutionary time (section 8.2) allows the inference that, averaged over time, birth rate equals death rate.
Parasitism and predation are major influences on population dynamics as they affect death rate in a manner that varies with host density. Thus, an increase in mortality with density (positive density dependence) contrasts with a decrease in death rate with density (negative density dependence). A substantial body of experimental and theoretical evidence demonstrates that predators and parasitoids impose density- dependent effects on components of their food webs, in a trophic cascade (see below). Experimental removal of the most important (“top”) predator can induce a major shift in community structure, demonstrating that predators control the abundance of subdominant predators and certain prey species. Models of complex relationships between predators and prey frequently are motivated by a desire to understand interactions of native predators or biological control agents and target pest species.
Mathematical models may commence from simple interactions between a single monophagous predator and its prey. Experiments and simulations concerning the long-term trend in densities of each show regular cycles of predators and prey: when prey are abundant, predator survival is high; as more predators become available, prey abundance is reduced; predator numbers decrease as do those of prey; reduction in predation allows the prey to escape and rebuild numbers. The sinusoidal, time-lagged cycles of predator and prey abundances may exist in some simple natural systems, such as the aquatic planktonic predator Chaoborus (Diptera: Chaoboridae) and its cladoceran prey Daphnia (Fig. 13.9).
Examination of shorter-term feeding responses using laboratory studies of simple systems shows that predators vary in their responses to prey density. Early ecologists’ assumptions of a linear relationship (increased prey density leading to increased predator feeding) have been superseded. A common functional response of a predator to prey density involves a gradual slowing of the rate of predation relative to increased prey density, until an asymptote is reached. This upper limit beyond which no increased rate of prey capture occurs is due to the time constraints of foraging and handling prey in which there is a finite limit to the time spent in feeding activities, including a recovery period. The rate of prey capture does not depend upon prey density alone: individuals of different instars have different feeding rate profiles, and in poikilothermic insects there is an important effect of ambient temperature on activity rates.
Assumptions of predator monophagy often may be biologically unrealistic, and more complex models include multiple prey items. Predator behavior is based upon optimal foraging strategies involving simulated prey selection varying with changes in proportional availability of different prey items. However, predators may not switch between prey items based upon simple relative numerical abundance; other factors include differences in prey profitability (nutritional content, ease of handling, etc.), the hunger-level of the predator, and perhaps predator learning and development of a search-image for particular prey, irrespective of abundance.
Models of prey foraging and handling by predators, including more realistic choice between profitable and less profitable prey items, indicate that:
- prey specialization ought to occur when the most profitable prey is abundant;
- predators should switch rapidly from complete dependence on one prey to the other, with partial preference (mixed feeding) being rare;
- the actual abundance of a less-abundant prey should be irrelevant to the decision of a predator to specialize on the most abundant prey.
Improvements can be made concerning parasitoid searching behavior which simplistically is taken to resemble a random-searching predator, independent of host abundance, the proportion of hosts already parasitized, or the distribution of the hosts. As we have seen above, parasitoids can identify and respond behaviorally to already-parasitized hosts. Furthermore, prey (and hosts) are not distributed at random, but occur in patches, and within patches the density is likely to vary. As predators and parasitoids aggregate in areas of high resource density, interactions between predators/parasitoids (interference) become significant, perhaps rendering a profitable area less profitable. For a number of reasons, there may be refuges from predators and parasitoids within a patch.
Thus, amongst California red scale insects (Hemiptera: Diaspididae: Aonidiella aurantii) on citrus trees, those on the periphery of the tree may be up to 27 times more vulnerable to two species of parasitoids compared with individual scales in the center of the tree, which thus may be termed a refuge. Furthermore, the effectiveness of a refuge varies between taxonomic or ecological groups: external leaf- feeding insects support more parasitoid species than leaf-mining insects, which in turn support more than highly concealed insects such as root feeders or those living in structural refuges. These observations have important implications for the success of biological control programs.
The direct effects of a predator (or parasitoid) on its prey (or host) translate into changes in the prey’s or host’s energy supply (i.e. plants if the prey or host is a herbivore) in an interaction chain. The effects of resource consumption are predicted to cascade from the top consumers (predators or parasitoids) to the base of the energy pyramid via feeding links between inversely related trophic levels. The results of field experiments on such trophic cascades involving predator manipulation (removal or addition) in terrestrial arthropod-dominated food webs have been synthesized using meta-analysis. This involves the statistical analysis of a large collection of analysis results from individual studies for the purpose of integrating the findings. Meta-analysis found extensive support for the existence of trophic cascades, with predator removal mostly leading to increased densities of herbivorous insects and higher levels of plant damage. Furthermore, the amount of herbivory following relaxation of predation pressure was significantly higher in crop than in non-crop systems such as grasslands and woodlands. It is likely that “top-down” control (from predators) is more frequently observed in man- aged than in natural systems due to simplification of habitat and food-web structure in managed environments. These results suggest that natural enemies can be very effective in controlling plant pests in agro-ecosystems and thus conservation of natural enemies (section 16.5.1) should be an important aspect of pest control.
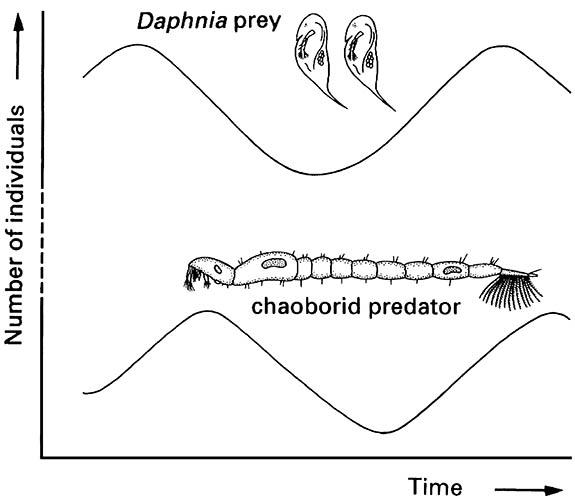